Selmer lab
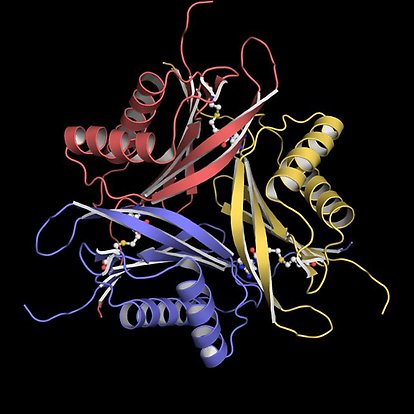
The goal of our research is to understand the structure and function of physiologically relevant macromolecules and macromolecular complexes. A main biological focus is translation in prokaryotes. Translation is a fundamental process in all living organisms, and increased understanding of this process can be used for development of new or modified antibiotics. We ask detailed questions regarding mechanisms of biogenesis, function and inhibition of the ribosome. We are also interested in how evolution acts on the atomic level of proteins. We use crystallography combined with biochemistry and biophysics as as tools to answer central biological questions regarding how macromolecules generate function.Our funding comes from the Knut and Alice Wallenberg foundation (RiboCORE 2012-2017, Evolution of new genes and proteins 2016-2021) and VR (Swedish research council) project grant and Environment grant "An integrated environment for Ribosome Research".
Popular science presentation
...
Research projects
The Selmer lab uses integrated structural biology to reach atom-to-organism understanding in two different areas, protein synthesis coupled to antibiotic resistance and evolution of protein structure and function. Below you find examples of our past and present research.
Ribosome biogenesis & antibiotic resistance
Translation is a fundamental process in all living organisms, and increased understanding of this process can be used for development of new or modified antibiotics. We ask detailed questions regarding mechanisms of biogenesis, function and inhibition of the ribosome and study molecular mechanisms of antibiotic resistance. We use X-ray crystallography and cryo-EM combined with other structural methods, biochemistry and biophysics to answer these questions.
Ribosomal RNA modification
Posttranscriptional modifications of rRNA play are important in ribosome biogenesis as well as in antibiotic resistance. We are interested in the structure and function of these enzymes, how they recognize their transiently available targets during bacterial ribosome assembly and in the function of the resulting modifications.
On-going work includes cryo-EM studies of ribosomal assembly intermediates and modification-deficient ribosomes.
Many RNA-methylating enzymes consist of a Rossman-fold methyltransferase domain in combination with a substrate-recognition domain. We have solved structures of 23S RNA modification enzymes RlmM (Punekar et al., NAR 2012) and RlmJ (Punekar et al., NAR 2013) and together with Anthony Forster, Uppsala University, clarified their substrate requirements. RlmJ in vitro can methylate a small RNA substrate mimicking H72 or 23S RNA, while RlmM requires a larger substrate and most probably recognizing RNA tertiary structure.
Crystal structure of erythromycin resistance protein
ErmE from the antibiotic producer Saccharopolyspora
erythraea (Stsiapanava et al., Sci Rep 2019). PDB entry: 6NVM
We have also solved the structure of erythromycin resistance enzyme ErmE (Stsiapanava et al., Sci Rep 2019), which N6A methylates 23S RNA nucleotide 2058 in the macrolide binding site, preventing drug binding. Despite showing relatively low level of sequence identity, we conclude that several different enzymes methylating the same position are showing highly similar structures.
To start to get insights into structures of early assembly intermediates that could act as MTase substrates during biogenesis of the 50S ribosomal subunit, we used cryo-EM to determine structures of LiCl core particles (Larsson et al., Biomolecules 2022). Salt-induced removal of ribosomal proteins from ribosomal subunits was early shown to produce particles that were assembly competent. We could now show that salt-washed particles are highly similar to in vivo assembly intermediates and visualize dependencies between complex tertiary RNA structures and RNA-protein interactions.
Elongation factor G and fusidic acid
Fusidic acid (FA) is an antibiotic that locks EF-G to the ribosome in translocation and ribosome recycling. The crystal structure of EF-G with FA on the 70S ribosome (Gao et al. Science 2009) shows how the drug binds in a pocket between domains I, II and III.
Staphylococcus aureus is one of the main targets of FA treatment. In collaboration with Suparna Sanyal, Uppsala University, we have worked out how EF-G from this organism evolves resistance. The crystal structure of EF-G from S. aureus (Chen et al., FEBS J 2010) allowed us to map all known resistance mutations on the structure. Only few mutations are close enough to the FA binding site to directly have an impact on drug interactions. Many of the mutations instead can be classified as affecting EF‐G–ribosome interactions, EF‐G conformational dynamics or EF‐G stability, in agreement with that FA only binds with high affinity to EF-G in a specific state on the ribosome. PDB entry: 2XEX
Biochemical studies and structures of S. aureus EF-G (Kiran Koripella et al., JBC 2012) allowed us to clarify the reasons for fitness loss of the F88L mutant and compensation in the double mutant F88L/M16I. PDB entries: 3ZZ0, 3ZZT, 3ZZU.
Fusidic acid resistance protein FusB. The C-terminal domain
is stabilized by a zinc ion (Guo et al., Open Biology 2012).
The clinically most prevalent type of FA resistance involves an EF-G binding resistance protein FusB (or its homologues FusB/C/D). We solved the crystal structure of FusB and mapped its binding site to domain IV of EF-G (Guo et al., Open Biol 2012). PDB entries: 4ADN, 4ADO. FusB-mediated rescue from FA inhibition during both translocation and recycling was confirmed in vitro in collaboration with the Sanyal group.
An on-going PhD project funded by the Uppsala Antibiotic Center aims to clarify the molecular details of this intriguing resistance mechanism.
Aminoglycoside modification enzymes
A common mechanism of aminoglycoside resistance is the covalent modification of drug molecules by resistance enzymes. We were intrigued by the bisubstrate specificity of ANT3"(9) aminoglycoside adenylyl transferase AadA and decided to elucidate how it achieves specificity for the two dramatically different substrates streptomycin and spectinomycin.
Using a combination of X-ray crystallography, bioinformatics and binding studies, we could show that the different active-site residues are essential for binding and modification of each substrate, while the catalytic reaction occurs at the same site. Crystal structures of AadA with ATP, magnesium and streptomycin explains why ANT3"(9) enzymes have characteristic sequence differences compared to ANT(9) enzymes that perform the same reaction on only spectinomycin (Chen et al., Acta Cryst D 2015; Stern et al., JBC 2018). A crystal structure of ANT(9) from Enterococcus faecium allowed visualization of the spectinomycin binding site, clarifying that spectinomycin and streptomycin extend in different direction from the catalytic site (Kanchugal P & Selmer, AAC 2020).
Structural understanding of protein evolution
We use structural biology in combination with other techniques to understand how evolution acts on protein structure to generate new functions or adaptation to new conditions. In a project funded by the Knut and Alice Wallenberg foundation, we study "novel" functional proteins, enzymes evolving additional or alternative functions and proteins involved in adaptation to new conditions, such as adaptation of herring to low salt conditions in the Baltic. These studies involves proteins from species ranging from bacteria to herring.
Bacteriophage-encoded SAM lyases
A bacteriophage-encoded SAM-degrading enzyme (SAMase) was first discovered in phage T3 in the 1960s and annotated as a SAM hydrolase. In a study led by Dan Andersson, several new phage-encoded SAMases with extremely low sequence similarity to the T3 SAMase were discovered based on their ability to rescue an ilvA knockout strain (Jerlström-Hultqvist et. al., Nature Ecology and Evolution 2018). Degradation of SAM was causing up-regulation of the methionine biosynthetic pathway, where the promiscuous activity of MetB provided rescue of isoleucine biosynthesis.
SAM lyase Svi3-3 in complex with the
substrate analogue SAH (Guo, Söderholm,
Kanchugal P, Isaksen et al., eLife 2021)
We have characterized the structure and mechanism of one of these SAMases, Svi3-3 (Guo, Söderholm, Kanchugal P, Isaksen et al., eLife 2021), originating from phage DNA isolated in Svandammen, Uppsala. In collaboration with Johan Åqvist, Dan Andersson, Adolf Gogoll and co-workers, we could show that Svi3-3 and T3 SAMase are SAM lyases and not hydrolases as stated since the 1960s. DFT calculations predicted that the enzyme provides a water-free active site that promotes an intramolecular lyase reaction, and this could be experimentally proven using TLC and NMR. PDB entries: 6ZM9, 6ZMG, 6ZNB
The SAMase from phage T3 was early found to co-purify with a protein from the E. coli host. In collaboration with Artem Isaev, Konstantin Severinov and coworkers we recently solved the cryo-EM structure of the complex between T3 SAMase and E. coli methionine synthase (MetK) (Andriianov, Triguis et al., Cell Reports 2023). Complex formation with MetK allows the small SAMase to reduce SAM levels by two mechanisms: direct degradation and inhibition of SAM synthesis. We show that both mechanisms counteract the bacterial BREX defence, and that MetK binding is more important than lyase activity.
We now aim to further characterize the SAM lyase family and their role in the arms race between bacteriophage and their host bacteria.
"Giant" surface proteins of Lactobacillus kunkeei
Lactobacillus kunkeei is the dominating bacterium in the honey gut of bees. In collaboration with the group of Siv Andersson, Uppsala University, we elucidate the structure and function of "giant" extracellular proteins of 3000-8000 amino acids to which L. kunkeei devotes a significant part of their genome.
Phosphoglucomutase 5 from Clupea harengus
In collaboration with the groups of Leif Andersson and Per Jemth, we have investigated the structure and function of PGM5 from Baltic and Atlantic herring (Gustafsson et al., Biomolecules 2020).
PGM5 in complex with glucose-1-phosphate
(Gustafsson et al.,Biomolecules 2020)
We have solved the first structure of PGM5, an intriguing protein that is homologous to the enzymatically active PGM1. In human, PGM5 has been shown to have a structural role, while PGM1 is a well characterised phosphoglucomutase.
The active site of PGM5 is highly similar to PGM1 and binds substrate in a close to identical manner. Yet, we could not detect any phosphoglucomutase activity suggesting that PGM5 also in herring is a structural protein and not an active enzyme.
PGM5 is one of the proteins where a single amino acid substitution has been linked to salinity and/or spawning time of herring. The surfaced-exposed location of this conservative mutation Ala330Val suggests that it may affect a protein-protein interaction rather than the intrinsic properties of PGM5.
Evolution of bifunctionality and specialization in HisA
In a fruitful collaboration with Wayne Patrick, now at Victoria University of Wellington, and Dan Andersson, Uppsala University, we have worked out the structural mechanism of how the amino acid biosynthetic enzyme HisA from Salmonella enterica can evolve bifunctionality to also take the role of TrpF and further specialize on either of the two functions. The initial structural characterization of HisA showed a two-step binding mechanism of substrate (Söderholm et al., JBC 2015). PDB entries: 5AHE, 5AHF, 5A5W.
Structural and biochemical characterization of a large set of real-time evolved HisA mutants (Newton et al., PNAS 2017) showed that bifunctionality is based on two distinct conformations of the active-site loops. Protein dynamics using NMR (Patrik Lundström, Linköping University) allowed us to prove the critical role of a single point mutation in providing the flexibility essential for bifunctionality. Interestingly, we observed two different mechanisms of how specialisation on the TrpF reaction evolved: stabilisation of the required active-site loop conformation and closing of the active site to prevent binding of the larger HisA substrate. Biochemistry and in vivo experiments showed that beyond ae activity threshold, improved enzymatic activity did not lead to increased growth rate. PDB entries: 5G1T, 5AC7, 5AC8, 5L9F, 5AB3, 5G1Y, 5L6U, 5G4E, 5G4W, 5G2I, 5G2W, 5G2H, 5G5I
Based on a serendipous finding in the previous study, we also solved the crystal structure of IGPS (or TrpC) from Pseudomonas aeruginosa, the enzyme catalysing the step after TrpF in tryptophan biosynthesis, and characterized its surprising substrate promiscuity (Söderholm et al., JBC 2020). Even though IGPS is classified as a decarboxylase, decarboxylation not completely essential. We showed that the enzyme in addition to its well characterized formation of indole-3-glycerol phosphate (IGP) from the natural substrate also can catalyse formation of the same product from decarboxylated substrate. PDB entry: 6Y88
Group members
Publications
Part of Scientific Reports, 2024
- DOI for Structures of the Staphylococcus aureus ribosome inhibited by fusidic acid and fusidic acid cyclopentane
- Download full text (pdf) of Structures of the Staphylococcus aureus ribosome inhibited by fusidic acid and fusidic acid cyclopentane
Part of Cell Reports, 2023
- DOI for Phage T3 overcomes the BREX defense through SAM cleavage and inhibition of SAM synthesis by SAM lyase
- Download full text (pdf) of Phage T3 overcomes the BREX defense through SAM cleavage and inhibition of SAM synthesis by SAM lyase
Structural Consequences of Deproteinating the 50S Ribosome
Part of Biomolecules, 2022
- DOI for Structural Consequences of Deproteinating the 50S Ribosome
- Download full text (pdf) of Structural Consequences of Deproteinating the 50S Ribosome
Structure and mechanism of a phage-encoded SAM lyase revises catalytic function of enzyme family
Part of eLIFE, 2021
- DOI for Structure and mechanism of a phage-encoded SAM lyase revises catalytic function of enzyme family
- Download full text (pdf) of Structure and mechanism of a phage-encoded SAM lyase revises catalytic function of enzyme family
Part of Journal of Biological Chemistry, 2021
- DOI for Structures and kinetics of Thermotoga maritima MetY reveal new insights into the predominant sulfurylation enzyme of bacterial methionine biosynthesis
- Download full text (pdf) of Structures and kinetics of Thermotoga maritima MetY reveal new insights into the predominant sulfurylation enzyme of bacterial methionine biosynthesis
Oligomerization and characteristics of phosphoenolpyruvate carboxylase in Synechococcus PCC 7002
Part of Scientific Reports, 2020
- DOI for Oligomerization and characteristics of phosphoenolpyruvate carboxylase in Synechococcus PCC 7002
- Download full text (pdf) of Oligomerization and characteristics of phosphoenolpyruvate carboxylase in Synechococcus PCC 7002
Structural Recognition of Spectinomycin by Resistance Enzyme ANT(9) from Enterococcus faecalis
Part of Antimicrobial Agents and Chemotherapy, 2020
Part of Biomolecules, 2020
- DOI for Structure and Characterization of Phosphoglucomutase 5 from Atlantic and Baltic Herring: An Inactive Enzyme with Intact Substrate Binding
- Download full text (pdf) of Structure and Characterization of Phosphoglucomutase 5 from Atlantic and Baltic Herring: An Inactive Enzyme with Intact Substrate Binding
Part of Journal of Biological Chemistry, p. 15948-15956, 2020
- DOI for Structure and kinetics of indole-3-glycerol phosphate synthase from Pseudomonas aeruginosa: Decarboxylation is not essential for indole formation
- Download full text (pdf) of Structure and kinetics of indole-3-glycerol phosphate synthase from Pseudomonas aeruginosa: Decarboxylation is not essential for indole formation
Crystal structure of ErmE-23S rRNA methyltransferase in macrolide resistance
Part of Scientific Reports, 2019
- DOI for Crystal structure of ErmE-23S rRNA methyltransferase in macrolide resistance
- Download full text (pdf) of Crystal structure of ErmE-23S rRNA methyltransferase in macrolide resistance
Part of Nature Ecology & Evolution, p. 1321-1330, 2018
Part of Journal of Biological Chemistry, p. 11481-11490, 2018
- DOI for Structural mechanism of AadA, a dual specificity aminoglycoside adenylyltransferase from Salmonella enterica
- Download full text (pdf) of Structural mechanism of AadA, a dual specificity aminoglycoside adenylyltransferase from Salmonella enterica
Structural and functional innovations in the real-time evolution of new (βα)8 barrel enzymes
Part of Proceedings of the National Academy of Sciences of the United States of America, p. 4727-4732, 2017
- DOI for Structural and functional innovations in the real-time evolution of new (βα)8 barrel enzymes
Two proofreading steps amplify the accuracy of genetic code translation
Part of Proceedings of the National Academy of Sciences of the United States of America, p. 13744-13749, 2016
Structure of AadA from Salmonella enterica: a monomeric aminoglycoside (3'')(9) adenyltransferase
Part of Acta Crystallographica Section D, p. 2267-2277, 2015
- DOI for Structure of AadA from Salmonella enterica: a monomeric aminoglycoside (3'')(9) adenyltransferase
- Download full text (pdf) of Structure of AadA from Salmonella enterica: a monomeric aminoglycoside (3'')(9) adenyltransferase
Part of Journal of Biological Chemistry, p. 24657-24668, 2015
Investigating Ribosome Conformations with Multi-Resolution Modeling
Part of Biophysical Journal, 2014
Part of PLOS ONE, 2014
- DOI for Resistance to beta-Lactam Antibiotics Conferred by Point Mutations in Penicillin-Binding Proteins PBP3, PBP4 and PBP6 in Salmonella enterica
- Download full text (pdf) of Resistance to beta-Lactam Antibiotics Conferred by Point Mutations in Penicillin-Binding Proteins PBP3, PBP4 and PBP6 in Salmonella enterica
Energetic pathway sampling in a protein interaction domain
Part of Structure, p. 1193-1202, 2013
- DOI for Energetic pathway sampling in a protein interaction domain
- Download full text (pdf) of Energetic pathway sampling in a protein interaction domain
Part of Acta Crystallographica. Section F, p. 1001-1003, 2013
- DOI for Purification, crystallization and preliminary X-ray diffraction analysis of the 23S rRNA methyltransferase RlmJ from Escherichia coli
- Download full text (pdf) of Purification, crystallization and preliminary X-ray diffraction analysis of the 23S rRNA methyltransferase RlmJ from Escherichia coli
Structural and functional insights into the molecular mechanism of rRNA m6A methyltransferase RlmJ
Part of Nucleic Acids Research, p. 9537-9548, 2013
- DOI for Structural and functional insights into the molecular mechanism of rRNA m6A methyltransferase RlmJ
- Download full text (pdf) of Structural and functional insights into the molecular mechanism of rRNA m6A methyltransferase RlmJ
Crystal structure of RlmM, the 2'O-ribose methyltransferase for C2498 of Escherichia coli 23S rRNA
Part of Nucleic Acids Research, p. 10507-20, 2012
- DOI for Crystal structure of RlmM, the 2'O-ribose methyltransferase for C2498 of Escherichia coli 23S rRNA
- Download full text (pdf) of Crystal structure of RlmM, the 2'O-ribose methyltransferase for C2498 of Escherichia coli 23S rRNA
Part of Journal of Biological Chemistry, p. 30257-30267, 2012
Ribosome engineering to promote new crystal forms
Part of Acta Crystallographica Section D, p. 578-583, 2012
Part of Open Biology, p. 120016, 2012
Tolerance of Protein Folding to a Circular Permutation in a PDZ Domain
Part of PLOS ONE, 2012
- DOI for Tolerance of Protein Folding to a Circular Permutation in a PDZ Domain
- Download full text (pdf) of Tolerance of Protein Folding to a Circular Permutation in a PDZ Domain
Staphylococcus aureus elongation factor G - structure and analysis of a target for fusidic acid
Part of The FEBS Journal, p. 3789-3803, 2010
Part of Journal of Biological Chemistry, p. 18051-18059, 2010
The structure of the ribosome with elongation factor G trapped in the posttranslocational state
Part of Science, p. 694-699, 2009
Crystal structure of the ribosome recycling factor bound to the ribosome
Part of Nature Structural & Molecular Biology, p. 733-737, 2007
Part of RNA, p. 817-823, 2007
People
Maria Selmer, Principal investigator,
Professor in Structural Biology, Distinguished University Teacher
Tel: 018-4714177 mobile: 070-1679761 Office: A7:2
Daniel Larsson,PhD, researcher
Fan Yang, PhD, postdoc
Shirin Akbar, PhD, postdoc
Silvia Trigüis, PhD student
Adrián González López, PhD student
Shruthi Krishnaswamy, Master student
Former group members
Eleni Kapidou, Summer student 2023
Jean-Rémi Cazette, Master student 2023
Henrik Cullhed, Master student 2022-2023
Maritha Johansen, Master student 2022-2023
Lukas Baumann, Erasmus student 2022-2023
Linnea Wikström, Project student 2022
Li Zhang, AT researcher 2020-2022
Emelie Oscarsson, Project student 2021
Emelia Karlsson, Project student 2021
Árni Thorlacius, Master student 2021
Sandesh Kanchugal Puttaswamy, PhD student 2014-2020 (PhD thesis), postdoc 2020
Robert Gustafsson, postdoc 2018-2020
Martin Schol, Master student 2019-2020
Annika Söderholm, PhD student 2013-2019 (PhD thesis), postdoc 2019
Josefin Ågren, Master student 2018-2019
Alex Taubert, Master student 2019
Alena Stsiapanava, researcher 2017-2019
Dennis Björklund, master student 2017-2018
Harald Bernard, Project student 2017
Ulrich Eckhard, researcher 2016-2017
Xiaohu Guo, PhD student 2010-2015 (PhD thesis), postdoc 2015-2016
Ana Laura Stern, Researcher 2014-2016
András Erdélyi, Project student and Master student 2015-2016
Roopa Kothapalli, postdoc 2014-2015
Kim Berit Bartels, project student autumn 2015
Sander van der Verren, Master student spring 2015
Maurice Diwo, project student spring 2015
Avinash Punekar, PhD student 2008-2014 (PhD thesis), postdoc 2014
Yang Chen, PhD student 2007-2013 (PhD thesis), postdoc 2013-2014
Shiying Wu, Postdoc 2012-2014
Kristina Bäckbro, Researcher 2008-2013
Zhe Zhang, Project student spring - summer 2012
Cha San Koh, Postdoc 2008-2011
Lisa Sophia Wolff, Project student autumn 2012
Levon Chant Halabelian, Project student summer 2011
Irina Trotsenko, Project student spring 2011
Ganesha Pandian Pitchai, Project student spring 2010
Hannes Beyer, Project student autumn 2009
Zhaolin Yang, Project student spring - summer 2009
Jendrik Hentschel, Project student spring - summer 2009
Aditya Mojumdar, Project student spring 2009
Christina Patlaka, Project student summer 2008
Chengcong Han, Project student July 2007 - June 2008
Ivaylo Dimitrov, visiting PhD student Nov 2007 - Feb 2008
Maria Bergquist, Project student spring 2007
Mats Ljung, Project student autumn 2006